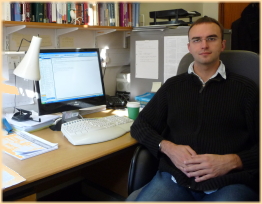
Dr Martin Galpin
Physical and Theoretical Chemistry Laboratory,
South Parks Road, Oxford, OX1 3QZ.
Tel: +44 [0]1865 285721
An overview of my research
I'm currently working on various theories of so-called 'quantum impurity' models, proposed originally to explain how the low-temperature behaviour of metals is affected by impurity atoms. The prototypical example is the Anderson impurity model, in which one considers just a single atom introduced into an otherwise perfect metal. Some twenty years after this model was conceived, its true low-temperature behaviour was eventually uncovered with the development of renormalization-group techniques (by future Nobel laureates Kenneth Wilson and Philip Anderson amongst others) and by the use of the Bethe ansatz. The model has since been studied using a number of other approaches including bosonization, conformal field theory, and the 'local moment approach' developed in Oxford by my supervisor Professor David Logan and coworkers.
The Anderson
model is difficult to analyze theoretically because it is a true
quantum 'many-body' problem. All the electrons in the host
metal interact with the impurity atom, and they do so in a correlated
fashion (rather like the way people trying to move through a crowd have
to take each other's movements into account in order to get anywhere).
Successful theories of the Anderson model cannot assume that each
electron moves independently of the others (in which case the problem
could be solved one electron at a time); instead, the motion of the
electrons as a whole must be considered explicitly. Moreover, the
electrons in the system move according to the laws of quantum
mechanics, and it turns out that even the quantum mechanical behaviour
of the helium atom (with only two electrons) cannot be calculated
exactly. The metallic hosts described by the Anderson model involve macroscopically
large numbers of electrons (i.e. typically of order 1023),
and hence it is necessary to use rather sophisticated many-body
techniques to tackle the problem.
The
eventual solution of the Anderson model led to the understanding of an
interesting phenomenon known as the Kondo effect.
Although there is no simple 'handwaving' picture that explains all its
subtleties, the basic idea is as follows. Consider a single atom such
as copper, which on its own has an unpaired electron in its outer
shell. If one were able to measure the magnetic moment of this single
free atom, one would obtain a finite value: the electron spin has to
point in some direction or other. But if the copper atom is instead
embedded as an impurity in a metal such as gold, the Kondo effect
essentially causes its magnetic moment to be reduced to zero (i.e 'quenched')
at low-temperatures by the conduction electrons of the host. The
process by which this quenching occurs is very complicated: in essence,
the unpaired electron on the impurity actually prefers to pair up with
a large number of the host electrons to form a complicated many-body
'singlet' state that shows no magnetic behaviour. This singlet state
has many interesting properties: for example, it leads to an increase
in the resistance of the host metal at low temperatures, rather than
the monotonic decrease that one would otherwise expect as the
vibrations of the metal atoms are gradually frozen out.
Recently, the
Kondo effect (and quantum impurity problems in general) have become
very popular due to advances in so-called 'quantum dot' fabrication
techniques. By trapping electrons in a small region of space which is
then 'wired up' to anelectrical circuit, the Kondo effect can be
studied experimentally in great detail. Indeed, many distinct Kondo
effects have been realised in such experimental devices, leading to a
range of interesting physical properties that may have important
consequences in designing novel electrical devices on the nanometre
scale.
My own research has been concerned with understanding a variety of Kondo effects in different systems. Over the last few years I have studied a model of two quantum dots coupled by an interdot Coulomb repulsion, which has recently become important in understanding the Kondo effects in quantum dots built inside carbon nanotubes. Such systems are interesting because the geometry of the carbon nanotube device can give rise to an unusual 'SU(4)' Kondo effect in which the electrons' spin and orbital motion becomes entangled. In our latest work in this area, my collaborators and I have shown how spin-orbit coupling can break this ideal SU(4) symmetry, thereby explaining a number of recent experimental observations.
In other work, I have been interested in the behaviour of quantum dots with additional internal 'degrees of freedom', as well as a model of a quantum dot sandwiched between semiconductors rather than metallic wires. It turns out that Kondo effects are not always possible in these more complex situations, leading to 'quantum phase transitions' (i.e. dramatic change in the nature of the system's ground state) with interesting physical consequences. Understanding these quantum phase transitions has formed a large part of my recent work, combining a number of theoretical approaches including the local moment approach and numerical renormalization group.